Harnessing nuclear energy
PDF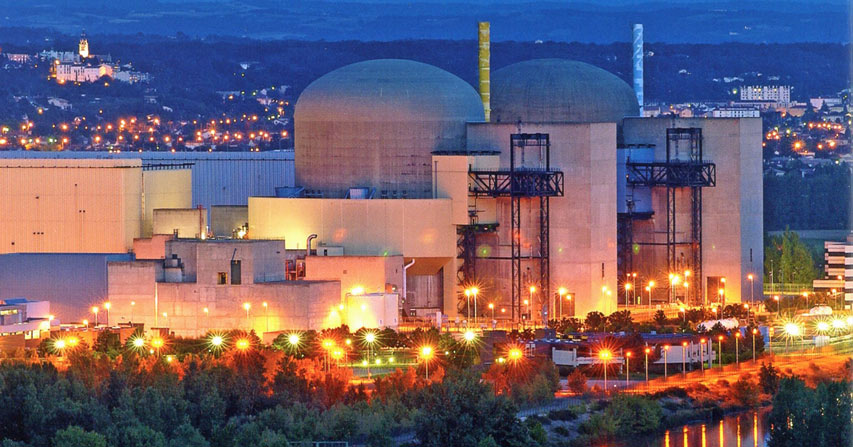
A nuclear reactor converts into electricity the heat produced by the fission of uranium nuclei. This electricity production can be adjusted according to demand. During normal operation, nuclear reactors are quite environmentally friendly, particularly with regard to greenhouse gases. Since the 1950s, reactors have evolved into “generations”, learning from the rare accidents to enhance their safety and robustness. Radioactive waste is produced in limited volumes, which allows for its containment in the very long term, but its management remains controversial.
In this article, we will describe the fission reaction that causes the energy used by about 450 nuclear reactors worldwide to produce 11% of the world’s electricity, the operation of these types of machines, and their positive and negative impacts on the environment. Before discussing this text, it is advisable to read the article “Radioactivity and nuclear reactions“.
1. Nuclear fission
This energy appears mainly in the form of kinetic energy from fragments that separate at high speed, but when those shake up neighbouring atoms, everything is transformed into heat (see “Energy“). We shall see that nuclear reactors called power reactors use this heat to produce electricity. An elementary chemical reaction typically releases an energy of a few electron volts (eV). A fission releases 200 million electron volts (200 MeV). The fission of one gram of uranium thus produces more energy than the combustion of one ton of oil.
After fission, if the new neutrons encounter other fissile nuclei, those may in turn split, giving rise to a chain reaction. If this chain develops exponentially, it very quickly releases an enormous amount of energy: this is the principle of atomic bombs. If the number of neutrons is kept constant, the power released is stable: this is what is achieved in a nuclear power plant.
It was Enrico Fermi who first observed (in 1934) that if neutrons were slowed down by elastic shocks on light nuclei (paraffin) before being directed towards a target material, the latter would start to absorb much more neutrons. This slowing is called moderation or thermalisation, because the neutron velocity decreases to the level that thermal agitation confers on the nuclei of the moderator material. This phenomenon plays a keyrole in the operation of most nuclear reactors, those called thermal reactors, as opposed to “fast neutron reactors” ( FNR ).
Some other heavy nuclei are not fissile, but after absorption of a neutron they disintegrate by beta radioactivity [1] and their resulting “grandson” is fissile. These nuclei, called fertile, are thorium 232Th which becomes uranium 233U, and uranium 238U which becomes plutonium 239Pu.
Now let us look at the fission fragments. Most are radioactive and decay more or less quickly, giving rise to other nuclei, often also radioactive. Fission products are the mixture of surviving fragments and the descendants of these fragments. The vast majority of fission products have no use and constitute the bulk of “high-level” radioactive waste (we will discuss their management below). In addition to fission products, waste also includes some heavy nuclei that come from non-fission neutron absorption: neptunium, americium and curium. They are called minor actinides (MA), and their long radioactive half-life[1] poses a specific problem that we will discuss later.
As a reactor produces energy, fission products accumulate in its core. When the chain reaction is stopped, these fission products continue to disintegrate, releasing significant residual power which is significant at first but decreases with time. A typical reactor thus releases 5% of its nominal power after 10 s of shutdown, 0.5% after one day, 0.3% after one week. The latter corresponds to 9 MW thermal for power plant producing 1000 MW in electrical power (3 times more thermal power). This phenomenon, specific to nuclear reactors, is undoubtedly the main safety problem: when the machine is switched off, the core must still be cooled for several weeks to avoid its melting, which is precisely what happened at Three Mile Island (United States) and Fukushima (Japan) during accidents that we will describe later.
2. Nuclear reactors
2.1. Power plant, reactor
We will keep the popular designation of power plant to designate a power generation unit, although the electrical companies refer more to a unit and often operate several units on a production centre. There are hydroelectric power plants, “conventional” thermal power plants whose energy comes from the combustion of coal, gas or, more rarely nowadays, heavy fuel oil, and nuclear power plants.
With the exception of simple gas turbines, thermal power plants convert liquid water into high-pressure steam in their boilers. This steam expands in a turbine, entraining its blades, then returns to a liquid state in a condenser whose tubes are fed by cooling water. The condensed water is then returned to the boiler for a new water-steam cycle. The axis of the turbine (often made up of several “bodies”) is fixed to that of an alternator whose rotation produces the electric current. All turbine bodies and the alternator are referred to as the turbo alternator unit. The electricity produced is sent to an electrical substation, a transformer that increases its voltage, before being sent to the high-voltage transmission grid (the higher the voltage, the lower the losses on the transmission lines).
A nuclear power plant is a thermal power plant whose energy that feeds the water-steam cycle comes from a fission chain reaction maintained in the core of a nuclear reactor.
2.2. nuclear fuel, core
By analogy with other thermal power plants, and although combustion plays no role, nuclear fuel is the fissile material that releases the desired heat. It must therefore contain fissile nuclei of uranium or plutonium. The most commonly used fuel is a sintered ceramic (agglomerated) from powdered oxide powder and formed into a cylindrical pellet.
The fuel is contained in assemblies with a dual function: on the one hand, they confine the various radioactive elements produced in the fuel, and on the other hand, they efficiently transfer the heat released by nuclear reactions to a heat transfer fluid (gas or liquid). This heat transfer feeds the power generation facility and in return it maintains the fuel at the desired temperature.
2.3. A controlled chain reaction
To maintain a chain reaction in a nuclear reactor, the number of neutrons produced in the core by fissions must at all times be exactly equal to the number of neutrons that disappear into or escape from the core. The ratio of production to disappearance is called the multiplication coefficient, noted K, and must therefore be strictly equal to 1, which is called criticality, and the reactor is called critical.
If this number K is less than 1, the neutrons disappear quickly, the chain reaction stops and so does the reactor: the core is said to be subcritical. On the other hand, if K is greater than 1, the number of neutrons will increase very quickly (and therefore so will the fissions, as well as the energy released in the core) and the chain reaction will “overreact”. It is then said that the heart is over-critical.
To keep the reactor permanently in a critical state (K = 1), neutron poisons or absorbents, composed of nuclei that absorb neutrons, are introduced (or removed) as required. We generally use 3 types of absorbents:
- moving bars, called control bars or clusters, that more or less penetrate into the core;
- absorbent products dissolved in the coolant and whose concentration can be varied over time. This is referred to as homogeneous poisoning.
- Absorbents dispersed in the fuel itself and gradually disappearing. They are called consumable poisons.
2.4. The key role of delayed neutrons
The time interval between two successive fissions during a chain reaction is excessively short (even the slowest neutrons still travel at 2 km/s!): there is no procedure to adjust the amount of poison in the core over this period. Fortunately, among the fission products, some have one extra neutron, but instead of getting rid of it by turning it into proton by beta disintegration (see “Radioactivity and Nuclear Reactions“), they simply eject it, after a delay that can be measured in minutes.
It is these delayed neutrons (as opposed to the prompt neutrons emitted during fission itself) that give the time needed to adjust poisons, such as raising or lowering control rods, and control reactor power. Without delayed neutron, there is no control of the chain reaction, and therefore no nuclear reactor.
The fission of a plutonium nucleus gives more neutrons (2.9 neutrons) than that of a uranium 235U nucleus (2.4 neutrons). On the other hand, the proportion of delayed neutrons is lower in plutonium fission (0.21% instead of 0.64%), which means that a plutonium core is more “nervous”.
3. The “generations” of nuclear reactors
The “first generation” refers to the initial proliferation of prototypes from the 1950s and 1960s, all different, now almost all stopped and at various stages of dismantling.
Generation II includes the reactor systems currently in operation, which supply 11% of world’s electricity. These reactors are robust, reliable and competitive. The vast majority of these reactors use ordinary water as coolant.
It is the Chernobyl accident, which we will describe below, that is at the origin of Generation III under construction: these reactors are required that if the major accident of total core meltdown occurs, the radioactivity remains confined in the site, i.e., in fact, inside the reactor building. The Evolutionary Power Reactor (EPR) of Franco-German design is the archetype of the Generation III reactor. It is a high-power (1650 MW) pressurised water reactor, highly protected and equipped with highly redundant backup systems that ensure its robustness and safety. The first two EPRs under construction in Finland and France experienced major construction difficulties, resulting in considerable delays and additional costs. The next two, recently achieved in China, do not have these problems because they benefit from the feedback of the first ones.
Finally, while Generation I is stopped, Generation II is exploited and Generation III is built, the “tiling” continues, and Generation IV is prepared with the aim of commercializing it around 2050.
Generation IV is expected to meet the requirements of a context that will be different from today. This is expected to lead to better use of fissile materials, more efficient management of long-lived radioactive waste, better resistance to proliferation [2], safety at least as high as that of Generation III, and the ability to open up to applications other than just the supply of electricity: desalination of seawater, heat production for industry, hydrogen production for the manufacture or improvement of synthetic fuels, etc.
The French RNR Superphénix, which operated from 1985 to 1997, was a precursor of Generation IV.
GIF, the Generation IV International Forum, which brings together 15 countries involved in nuclear technology, has sketched out the composite profiles of 6 “desirable” reactors to guide research and development, some of which will be ready for industrialization by the deadline.
4. The controlled nuclear fusion

When a heavy nucleus is fissioned, the loss of mass of the components releases considerable energy. Similarly (or conversely), the fusion of two very light nuclei leads to a loss of mass and an even greater release of energy (in terms of the mass of the components). This is the type of reaction that occurs in the core of stars (Figure 4).
Fission and fusion were both discovered in 1938, but while the first fission generator reactor was commissioned in 1954, the use of fusion to produce electricity is still a distant prospect today.
The problem is that the light cores in question have a positive electrical charge and therefore repel each other. At the heart of the stars, the gigantic forces of gravity solve this problem, but on Earth we must find another method. In H-bombs, it is the intense radiation of a “conventional” atomic bomb that initiates the fusion: it is not transferable to controlled electricity production.
Most hopes are based on the very high temperature (100 million degrees) of a deuterium and tritium plasma, two hydrogen isotopes whose nucleus contains one or two neutrons respectively [3]. The speeds of the nuclei at these temperatures allow electrostatic repulsion to be overcome. Of course, at this temperature, this plasma must have no contact with a material wall: it is confined by a combination of magnetic and electric fields in a machine called a tokamak. ITER, the most powerful of these machines, has been under construction in Cadarache since 2005 in a very multinational setting. Around 2027, ITER should produce its first deuterium-tritium plasma: by then the equivalent for the fission of the Fermi experiment in December 1942 will have been more or less reached for fusion.
5. Nuclear energy and the environment
5.1. Greenhouse gases
5.2. Gaseous effluents and liquid discharge
Excluding accidents, nuclear power plants are characterized by very low levels of radioactive releases to the environment, which are closely monitored. The total amount of radioactivity released into the atmosphere from a nuclear power plant is less than that released from a coal-fired power plant due to the uranium and thorium impurities contained in it.
Before the systematization of the treatment of certain well-known mineral waters by passage over ion exchange resins, they would have been too radioactive to be authorized as liquid discharges from a power plant.
5.3. Thermal emissions
At the exit of any thermal power plant, nuclear or other, the water is discharged at a higher temperature than at the entrance. For seaside power plants, this is not a problem. For power plants cooled by rivers, the temperature difference is limited by regulation to a level that does not risk disturbing aquatic fauna. This often leads to not releasing the water into the environment without having cooled it by evaporation in these large air coolers, the plume of which can be identified from a distance (plume due to the condensation in the atmosphere of this evaporated river water). In France, these towers are associated with nuclear power plants, but in Germany, these same towers most often report coal-fired power plants.
5.4. Radioactive waste
While effluents and discharges are diluted into the environment due to their minimal radioactivity, radioactive waste is, by contrast, concentrated and immobilized in matrices appropriate to its nature. These solid packages are stored under control until they are stored under conditions that ensure their containment for as long as they present a danger.
A radioactive substance is a substance that contains radioelements whose quantity or concentration requires protective measures. Radioactive waste is radioactive material for which no use is planned or envisaged. In France, the ultimate management of waste is carried out by ANDRA, the French National Agency for Radioactive Waste Management, under the supervision of ASN, the French Nuclear Safety Authority.
For radioactive waste, the usual French classification is based on two important parameters to define the appropriate management method: the level of activity, which corresponds to the number of disintegrations per unit of time of the radioactive elements contained in the waste and the radioactive half-life of these radioelements. In particular, a distinction is made between waste whose radioactivity comes mainly from radioelements with a period of less than 31 years (so-called short-lived waste) and waste whose radioactivity comes mainly from radioelements with a period of more than 31 years (so-called long-lived waste).
- Very short-lived waste, mainly from the medical or research sector, is stored at their site of use for the duration of its radioactive decay.
- Very low level waste (VLW), less than 100 Bq/g (20 times the radioactivity of granite) is stored on the surface in duly authorized ANDRA sites. They are mainly derived from the operation, maintenance and dismantling of nuclear power plants (scrap metal, rubble, concrete), fuel cycle installations and research centres.
- Long-lived low-level radioactive waste (LLW) will be subject to a more stringent containment procedure. These are mainly graphite waste (dismantling of old power plants) and radioactive waste (radioactive ores).
- Long-lived intermediate level waste (LLW) and high level waste (HLW) are encapsulated in stainless steel containers that must be stored at great depth. They mainly come from spent fuel after processing. HA waste is integrated into a glass matrix before encapsulation to limit the heating produced by its radioactivity.
For the ultimate management of radioactive waste, Great Britain and Japan have chosen the same options as France and China are about to do the same, Sweden and Finland have chosen the geological storage of spent fuel without treatment, and most other countries are storing spent fuel pending a decision.
6. Accidents at nuclear facilities
6.1. The INES gravity scale
Since the beginning of the nuclear era, nuclear reactors have suffered very few accidents (INES level >3), here is the list:
We will only mention here the most well-known ones, as well as the lessons that have been learned.
6.2. Three Mile Island, TMI, March 29, 1979
Deceived by ambiguous instrumentation, the operators of this American reactor transformed a minor incident into a serious accident through their harmful interventions: a third of the core melted, the almost new reactor was taken out of service, and cleaning took 14 years. The containment withstood and the releases of radioactivity were minimal: there were no casualties, but the accident received considerable media coverage and the American public was durably traumatized.
The main lesson of TMI is that safety does not only depend on the quality of the machine, but also on the machine/operator pair. Many improvements have been made to nuclear reactors around the world to take into account the “human factor” and share feedback.
6.3. Chernobyl, 28 April 1986
The RBMK is a particular type of Soviet reactor designed to produce electricity but also plutonium for nuclear weapons. The functioning of RBMK showed instabilities at some regimes. To carry out a safety experiment, the operators deliberately violated a series of instructions and caused the chain reaction to run wild and a sudden increase in highly destructive power. The resulting steam explosion destroyed a quarter of the core and blew the roof off the building. In the open air, the graphite in the core ignited: the fire that lasted about ten days sent volatile radioactive products into the stratosphere, which, following the jet streams, flew over all of Europe, randomly contaminating its territory according to precipitation and the path of radioactive aerosols.
About 50 firefighters and operators died from acute radiation, and about 20 of the thousands of children suffering from thyroid cancer caused by the radioactive iodine released by the accident died. Among the “liquidators” [4] and the inhabitants of the neighbouring town, Pripyat, there may be up to 4000 premature deaths from induced radiation cancer, but the health damage due to evacuation is much greater.
The main lesson of Chernobyl was that such contamination is simply not tolerable. As a result, reactor designers have launched so-called third-generation power plants (EPR, AP 1000, etc.) on the basis of a very demanding criterion: even complete core meltdown must not lead to the long term evacuation of neighbouring populations.
6.4. Fukushima, 11 March 2011
While the two previous accidents were mainly man-made, the one in Fukushima was caused by a natural disaster. An exceptional earthquake (force 9) and the very powerful tsunami it triggered devastated the northeast coast of Honshu, Japan’s main island, killing 20,000 people. 14 nuclear reactors were spread over four sites in the devastated area, including 6 in Fukushima Daiichi. The earthquake caused the reactors to shut down automatically, but it also destroyed the high-voltage lines that connected the Fukushima Daiichi site to the grid. The 13 emergency diesels supplying the site’s reactor backup systems started immediately, as planned. But the tsunami then drowned 12 of them, as well as the electrical panels and batteries, making it impossible to evacuate the residual power (see section 1).
The cores of the three reactors (out of six) that were operating at Fukushima Daiichi during the earthquake overheated, the fuel sheaths reacted with water vapour to produce large quantities of hydrogen that caused explosions, and the cores melted. A fourth shutdown reactor was also damaged.
The first radioactive releases to the atmosphere were blown by the wind towards the Pacific where they diluted, but on the night of March 15 the wind shifted and a corridor about 50 km northwest of the site was heavily contaminated.
Thanks to early evacuation measures, local populations have received very little irradiation: we do not expect to detect a health effect of radiation beyond the background noise. On the other hand, the evacuation caused the death of sick or elderly people.
The Fukushima accident validated most of the options of generation 3, but illustrated the vulnerability of previous reactors: new measures to strengthen the robustness of backup systems are underway as well as an improvement of crisis management systems (for example, the creation by the company Electricite de France of the rapid action force FARN capable of supporting or even replacing operators during an accident).
The Fukushima accident is also teaching us a lot about the decontamination of the territories to allow the evacuated populations to return.
7. Treatment and natural decline of radioactive pollution.
But the nature of the soil also plays an essential role in the areas contaminated by the Chernobyl accident: cesium sinks quickly into clay soils whose surface radioactivity disappears as a result, while it is permanently recycled by peat. In villages in Ukraine or Belarus, people have learned to avoid certain “hot” areas but know that they can safely go about their business outside these areas.
While the evacuated area around Chernobyl remains prohibited, 29 years later, the Japanese are doing everything possible to allow the population to return as soon as possible, and some localities were reopened four years after the accident. They implement many decontamination methods:
- Cleaning of roofs – passage of walls to Karcher
- Scrape the soil over 5 cm
- Deep ploughing (> 30 cm)
- Remediation by certain plants
- Clearing in the vicinity of dwellings, etc.
These methods have proven their effectiveness but generate large quantities of waste to be stored. Forests remain very difficult to decontaminate, but no one stays there all the time. As for the impact on natural biodiversity, it seems limited and species-dependent[6], with the harmful effects of radiation being offset by the reduction of pressure due to human activities.
References and notes
Image cover. Saint Alban power plant (document Areva/Y. Geoffray)
[1] See definition in the article “Radioactivity and nuclear reactions“.
[2] To reduce the risk of diversion from civil nuclear power to military applications
[3] The words “plasma” and “isotope” are explained in the article “Radioactivity and nuclear reactions“.
[4] This is the name given to the people who intervened on site to decontaminate the site and build the famous “sarcophagus” to confine the entire plant
[5] Each radioactive atom is characterized by its specific “period”, after which time half of the atoms have disintegrated.
[6] http://www.irsn.fr/FR/connaissances/Installations_nucleaires/Les-accidents-nucleaires/accident-fukushima-2011/fukushima-2016/Documents/IRSN_Fukushima-Tchernobyl_faune-flore_201603.pdf.
The Encyclopedia of the Environment by the Association des Encyclopédies de l'Environnement et de l'Énergie (www.a3e.fr), contractually linked to the University of Grenoble Alpes and Grenoble INP, and sponsored by the French Academy of Sciences.
To cite this article: BARRÉ Bertrand (February 5, 2019), Harnessing nuclear energy, Encyclopedia of the Environment, Accessed July 27, 2024 [online ISSN 2555-0950] url : https://www.encyclopedie-environnement.org/en/physics/controlling-nuclear-energy/.
The articles in the Encyclopedia of the Environment are made available under the terms of the Creative Commons BY-NC-SA license, which authorizes reproduction subject to: citing the source, not making commercial use of them, sharing identical initial conditions, reproducing at each reuse or distribution the mention of this Creative Commons BY-NC-SA license.