Geothermal energy, a source of green energy under our buildings
PDF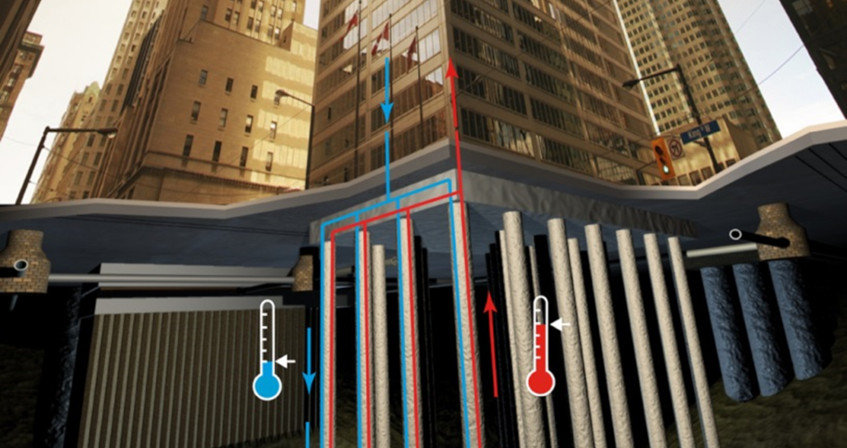
Energy geostructures are a modern application of low enthalpy geothermal systems (i.e. with low temperature differentials), with the major advantage of reducing initial installation costs compared to standard systems. The principle consists in setting up a network of pipes inside the reinforcement cages inside the concrete structures. A heat transfer fluid, which circulates in the pipe network, exchanges heat with the ground through the concrete. A heat pump adapts the temperature from the ground to that required to heat or cool buildings. The efficiency of the system must meet both energy (heat or cold production) and geotechnical-structural (limited additional stresses and displacements) criteria, which are highly dependent on the local conditions of the site hosting the construction.
In a world where energy needs are constantly increasing and where the research for green, local and renewable energy sources is becoming increasingly important, energy geostructures are perfectly suited. They represent an innovative and promising alternative for heating or cooling buildings and infrastructures.
1. What is an energy geostructure?
Geostructures, i.e. structures in contact with the ground can be used to exchange heat with the ground [1]. More precisely, the term geostructures includes piles for deep foundations, retaining walls, tunnel lining segments, etc. The heat exchange between the ground and these concrete structures is ensured by a system of tubes arranged inside the structure and within which a heat transfer fluid circulates.
The principle is that of shallow geothermal energy: thanks to the fact that the subsoil temperature remains constant throughout the year (except for the first 5 to 8 m), this temperature will be higher than that of the external air in winter and lower in summer. This temperature difference allows heat to be extracted from the ground to the building during the winter (for heating purposes) and injected from the building to the ground during summer (for cooling purposes). This falls into the category of the so-called “low enthalpy” geothermal systems, i.e. systems that involve the first 100 m of ground and work with temperature variations of around 10 to 20 degrees Celsius. To adapt the temperature coming from the heat exchangers to that required to heat or cool buildings, these systems are connected to a heat pump (read Geothermal: a significant source of energy).
1.1. Operating modes
The system can be used for both heating and cooling of buildings (dual mode) as well as only for heating or only for cooling (single mode). The choice of the operating mode depends, among other things, on the local groundwater flow conditions.
- In the case where groundwater flow is zero or very low, a thermal recharge of the soil is necessary in order to keep the temperature constant over the long term. In this case, it is strongly recommended to use the system in dual mode to ensure its efficiency.
- If, on the other hand, the soil is sufficiently permeable (sand) and subjected to a groundwater flow greater than 0.5 or 1 m per day, the soil temperature is automatically rebalanced and a simple mode is possible [2].
On the other hand, if in the first case (no ground water flow) seasonal heat storage is feasible, in the second one (significant ground water flow) this is not possible and extraction is naturally decoupled from injection.
1.2. Technology diffusion
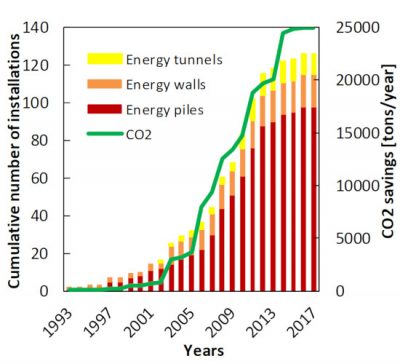
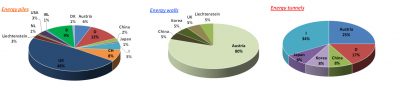
Energy geostructures have also been recently introduced in the United States and Asia. An example is the Shanghai Tower in China. It is the tallest tower in China with a height of 630 m, it rests on 2000 piles of 86 m in length and 1 m in diameter, about a hundred of which are equipped as energy piles.
1.3. Some examples of this are
In Europe, many projects have already been carried out in schools, private buildings or public buildings.
1.3.1. Energy piles
One of the most documented examples is probably the Terminal E at Kloten Airport (Zurich), which is operational since 2003. Designed by Amstein and Walthert AG, in partnership with ARGE ZAYETA, it is built on a clay and silty soil and its foundations consist of 350 drilled piles 27 m long and with a diameter between 90 and 150 cm. Of these, 306 are equipped as energy piles and provide 70% of the terminal’s heat requirements [4].The cooling of the building is also ensured by energy piles, 53% by free cooling and 15% by using the heat pump. In this specific case, the additional cost due to the instrumentation of the piles represented 0.3% of the building cost, which was paid back in 8 years.
1.3.2. Energy walls
Another classic example is the Lainzer Tunnel in Vienna, an energy tunnel built by the cut-and-cover method, where the excavation is first dug in the open air and then covered by concrete slabs. In this case, the heat is exchanged with the ground using the side walls, made of piles 20 m long and 120 cm in diameter. One out of three piles is equipped as an energy pile, which represents a total of 59 heat exchanger piles.
Also in Vienna, 4 sections of the U2 metro line are equipped with energy piles, invert and walls, for a total production of 449 kW of heating power and 231 kW of cooling capacity.
1.3.3. Energy tunnel
Energy tunnels are today the least developed application of this technology, despite their remarkable heat exchange potential. Indeed, the geothermal activation of tunnel linings (carried out either by mechanical excavation using a tunnel boring machine or conventional digging with a mechanical shovel) involves a larger volume of soil and therefore a generally larger exchange surface than piles.
The first example of an energy tunnel was built in Austria: the Janbach Tunnel. More recently, a full-scale experimental test was realised in the Turin metro tunnel in Italy.
1.4. Advantages
1.4.1. Economic
The main advantage of the installation of energy geostructures over other conventional geothermal systems is that structural elements that are necessary for the structural stability and thus would be constructed in any case are use also for energy purposes. This results in a reduction of the initial installation costs. Experience shows a payback period included between 4 and 8 years, the initial investment being in the order of 2 to 4% of the cost of the work.
1.4.2. Environmental issues

2. Energy efficiency
Figures 4, 5 and 6 summarize the heat exchange potential measured in operational energy geostructures, presented in Watt per metre of pile length and Watt per square metre of wall and tunnel surface, respectively.
2.1. Energy piles
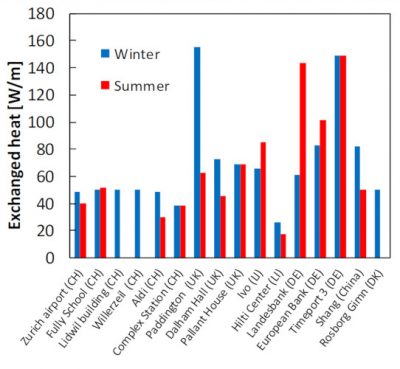
2.2. Energy walls and tunnels
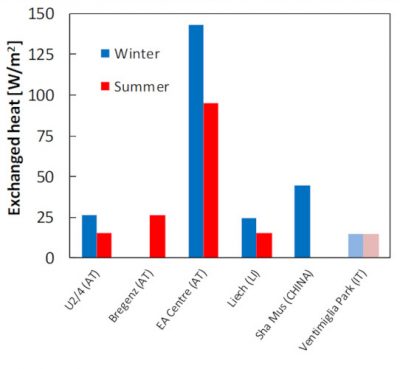
The numerical and analytical analysis of these structures is less straight forward due to the substantial difference with respect to geothermal probes. The main difference is represented by the boundary conditions on the excavation sides (for example inside a car park or tunnel or station). This obliges to take into consideration the heat exchange with the internal air as well. Moreover, the limited number of monitored cases makes things more complicated.
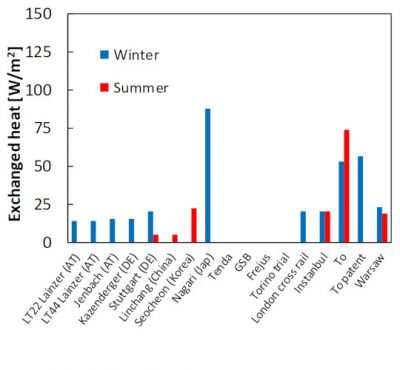
3.Geotechnical aspects
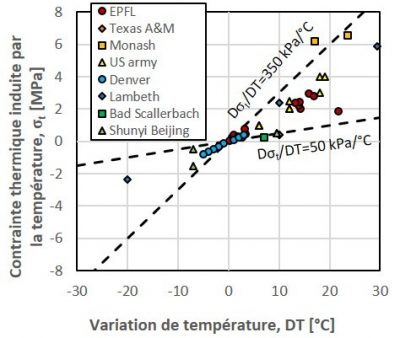
In other words, can the use of geostructures as ground heat exchangers induce additional displacements or reduce their load-bearing capacity (i.e. their ability to support applied loads such as the weight of a building)? Once again, the experimental and numerical results available concern mainly piles.
As a first approximation, a pile can be schematized as a vertical homogeneous bar. If we imagine heating it when it is completely free to deform, it will expand with respect to its center according to its coefficient of thermal expansion. To have an order of magnitude, let us consider a 30 m long pile, free to deform, and made of concrete, which has a coefficient of thermal expansion of about 10-5 °C-1. A temperature variation of 30 °C would induce a displacement of 4.5 mm (0.15 mm/°C) symmetrically at the top and at the bottom of it (and therefore at the head of the pile).

Of course, these are two extreme cases. The reality is a in between them, but overall one could say that the more the pile will be free to expand, the more there will be a significant displacement at the head and the less thermal stress and vice versa. Similar considerations can be made for cooling.
Figures 7 and 8 show the stress and displacement values induced by temperature variations, measured in full-scale tests carried out on energy piles. In practice, they can be used to have an order of magnitude of the effects that temperature changes induce on the geotechnical behaviour of real energy piles. It is important to note that, even if the thermal stresses look high, they remain well below the concrete strength.
4. Messages to remember
- Energy geostructures represent a green, renewable and local source of energy for heating and cooling of buildings.
- The main advantage with respect to other conventional closed geothermal systems (probes) is the significant reduction in initial installation costs, this reduction being due to the use of geostructures that would be constructed anyway.
- This technology is still under development, but the number of structures and buildings based on heat exchanger piles is increasing exponentially in Europe without any slowdown being expected at present.
- The application to energy walls and tunnels is less developed, but many projects are ongoing to better understand their behaviour and energy efficiency.
Notes and references
Cover image. Laloui, L., and Di Donna, A. 2013. Energy geostructures: innovation in underground engineering. ISTE Ltd and John Wiley & sons Inc. [Source: © EPFL-LMS /M. NUTH 2010 ]
[1] Laloui, L., and Di Donna, A. 2013. Energy geostructures: innovation in underground engineering. ISTE Ltd and John Wiley & sons Inc.
[2] AIS. 2015. Use of ground heat by concrete foundation and support structures. Guide for design, implementation and maintenance. Swiss Society of Engineers and Architects. SIA DO 190 documentation.
[3] Di Donna, A., Barla, M., M., and Amis, T. 2017. Energy geostructures: a collection of data from real applications. In 15th IACMAG, Wuhan, China.
[4] Pahud, D. 2002. Geothermal energy and heat storage. SUPSI – DCT – LEEE Laboratorio di Energia, Ecologia ed Economia: 1-133.
The Encyclopedia of the Environment by the Association des Encyclopédies de l'Environnement et de l'Énergie (www.a3e.fr), contractually linked to the University of Grenoble Alpes and Grenoble INP, and sponsored by the French Academy of Sciences.
To cite this article: DI DONNA Alice (January 5, 2025), Geothermal energy, a source of green energy under our buildings, Encyclopedia of the Environment, Accessed April 5, 2025 [online ISSN 2555-0950] url : https://www.encyclopedie-environnement.org/en/soil/geothermal-energy-source-green-energy-buildings/.
The articles in the Encyclopedia of the Environment are made available under the terms of the Creative Commons BY-NC-SA license, which authorizes reproduction subject to: citing the source, not making commercial use of them, sharing identical initial conditions, reproducing at each reuse or distribution the mention of this Creative Commons BY-NC-SA license.