Environmental constraints and oxidative stress in plants
PDF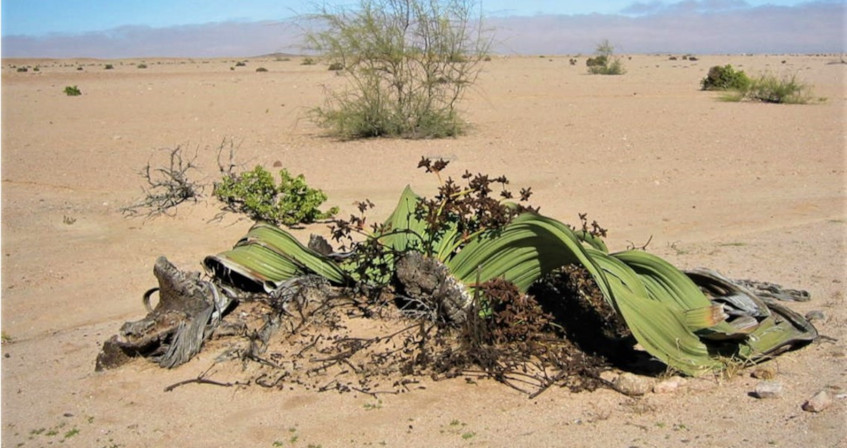
Plants cannot move and are therefore particularly exposed to environmental fluctuations and external attacks. Environmental conditions are very rarely optimal, leading to the concept of stress, which impacts plant growth and development. Environmental stresses include biotic stresses, caused by a living organism, and abiotic stresses, caused by environmental factors. Recent data in cell and molecular biology show that a number of stress response mechanisms are shared by plants and animals, with the cell being the common point, and even the common target, of all these living beings. In particular, toxic oxidative molecules, present during abiotic stresses, play similar roles in animal and plant cells. This article focuses on the abiotic stresses suffered by plants, which have been led to develop a particularly complex response system in order to adapt and survive.
1. Abiotic stresses
The word stress designates the aggression that a living organism undergoes and its reaction to the resulting state of disturbance. Etymologically, the word “stress” is borrowed from the same English word, but it originally comes from the Old French “déstresse”, which corresponds to distress.
Abiotic stresses [1] caused by a wide variety of environmental factors independent of living beings include the following (Figure 1):
- Temperature:
– Periods of frost (especially late frost)
– Too high temperatures (especially over long periods, which is happening more and more often, in connection with climate change); (See Effects of temperature on photosynthesis) - Water:
– Excess of water (flooding leading to root asphyxia)
– Lack of water causing droughts (See Water needs of plants : how to satisfy them ?) - Solar radiation: excess of light, UV (See Cellular impact of solar UV rays)
- Nutrient deficiencies: mineral elements (especially magnesium), nitrogen
- Soil salinity: excess of sodium and chlorine ions (See focus on Land salinization)
- Soil pollution: heavy metals (cadmium, lead, mercury, copper, aluminum, …)
- Atmospheric pollution: (See What is the impact of air pollutants on vegetation?)
– Primary pollutants: sulfur dioxide (SO2), nitrogen oxides (NOx), ammonia (NH3), volatile organic compounds (VOC)
– Secondary pollutant: ozone (O3). This gaseous compound is a photochemical pollutant. It results from interactions, in the presence of UV, between oxygen O2, nitrogen oxides (NO and NO2) and volatile organic compounds (VOC). The concentration of this highly phytotoxic compound has increased considerably in the troposphere between the 19th century and the 2000s. Currently, its annual average concentration is leveling off in the USA and Europe but continues to increase in Asia. However, acute concentration peaks occur over short periods of time and can cause plant damage [2].
– Carbon dioxide (CO2): This compound occupies a special place and its presence in the list of air pollutants may be surprising. Indeed, CO2 is the essential carbon source for plant growth (photosynthesis). However, the considerable increase in its content in the air since the end of the 19th century (its concentration has risen from 290 ppm at the beginning of the Industrial Revolution to nearly 420 ppm today), raises questions about the ability of plants to regulate this increased carbon input. It seems that the increase in CO2 results in a growth of the leaf surface allowing a proportional increase in photosynthesis [3]. However, at the scale of terrestrial vegetation growth, other constraints, such as drought, temperature increase and/or disruption of the carbon/nitrogen balance, could interfere with this relationship [4].
The adverse effect of abiotic stresses on crop yields has been known for almost half a century. The most incriminated abiotic stress is drought [5], although decreases in productivity have also been attributed to the presence of air pollutants [6].
- The interaction between the increase of CO2, which is a priori beneficial, and that of ozone, whose toxicity is obvious [7].
- Drought combined with the presence of ozone, both of which can be detrimental to plant development [8].
In the latter case, the way in which the two stresses occur over time can change their interaction from synergistic (coordinated action) to antagonistic (opposite effect). For example, the risk of plant damage is greater when ozone pollution occurs in the spring before the drought begins, compared to an early or simultaneous drought with ozone pollution, which would close the stomata and reduce the penetration of the pollutant.
Plants are able to develop mechanisms of perception and response to environmental stresses, allowing them to defend themselves by showing a certain adaptation. However, if the stress intensifies and exceeds the plant’s defensive capacities, the plant may die.
What is remarkable is that each of the various environmental constraints that plants must face results in the same type of stress: oxidative stress, expressed at the cellular level. Oxidative stress results from an imbalance between the production, or rather the overproduction, of reactive oxygen species (ROS) and cellular antioxidant capacities [9] (Figure 1).
2. Reactive oxygen species (ROS)
2.1. Chemical nature and mechanisms of ROS production
ROS are central to oxygen metabolism and the appearance of aerobic living organisms. Oxygen appears progressively in the Earth’s atmosphere about 3 billion years ago and then increases strongly around 2.3 billion years ago thanks to the multiplication of photosynthetic organisms. In its most stable state, oxygen exists in the form of oxygen, O2. Aerobic living organisms had to adapt to the presence of oxygen, by consuming it. Oxygen is essential to the respiration of these organ
- O2-, superoxide ion;
- HO, hydroxyl radical;
- HO2, perhydroxyl radical.
There is also 1O2, singlet oxygen [ii] and a very important non-radical form, hydrogen peroxide H2O2 [10] (Figure 2).
Finally, RO2–, peroxyl radical [iii] and RO–, alkoxyl radical [iv] are secondary radicals involving organic compounds R oxidized by 1O2 or HO–.
- Oxygen, produced by photosynthesis, receives energy from light-excited chlorophyll (triplet [iii] 3Chl*state), and gives rise to singlet oxygen 1O2 (Figure 3).
- Electrons available from intracellular biochemical reactions can react with oxygen to form superoxide ions [v], O2 –..
- The superoxide ion is relatively easily converted to hydroperoxide H2O2 by the action of the enzyme superoxide dismutase (SOD).
- The hydroperoxide is then transformed :
- In the presence of ferrous ions, it gives the hydroxyl radical HO• during the Fenton reaction [vi];
- It can also be broken down by catalases (CAT), enzymes that protect the cell from the oxidizing and harmful action of hydroperoxide (see 2.4).
- In turn, the superoxide ion can produce the perhydroxyl radical HO2. by protonation.
2.2. Sites of production of reactive oxygen species
ROS are normally produced in all cellular compartments, plastids (chloroplasts in aerial parts), mitochondria, peroxisomes/glyoxysomes, cytosol, plasma membrane, apoplast (cell wall) (Figure 4). Chloroplasts are the main source of ROS in photosynthetic tissues. Mitochondria are important ROS producers especially in non-photosynthetic tissues.
- Chloroplasts
– Singlet oxygen 1O2 is produced from oxygen delivered during photochemical reactions and which captures energy from excited 3Chl* at photosystem II.
– The formation of 1O2 is favored by a limitation of CO2 entry following the closure of the stomata, often observed during stress. The decrease of CO2 causes a slowing down of biochemical reactions resulting in a lower demand of photochemical reaction products and a concomitant electronic bottleneck (over-reduction).
– At the level of photosystem I, oxygen captures electrons to give the superoxide ion O2–. It can be further transformed into another ROS, the hydroperoxide H2O2 with the help of enzymes, the superoxide dismutases (SOD) (see paragraph 2.4).
- Mitochondria
In mitochondria, during respiration, electron leakage occurs naturally in the respiratory complexes, leading to the production of superoxide ions O2–. They can be converted by a SOD into H2O2.
- Peroxisomes
In peroxisomes [vii] the main source of ROS is the reduction of glycolate [viii] to glyoxylate by glycolate oxidase which uses O2 to give H2O2. O2– ions can also be produced during the operation of the enzyme xanthine oxidase and transformed into H2O2 by a peroxisomal SOD.
- Glyoxysomes
In glyoxysomes [ix] the β-oxidation [11] of fatty acids produces hydroperoxide H2O2.
- Plasma membrane & apoplast
The plasma membrane contains an NADPH oxidase [12] that delivers into the apoplast [x] O2.- superoxide ions formed from oxygen. In the apoplast, hydroperoxide is found which comes either from (i) the transformation of superoxide ions by a SOD, (ii) the action of peroxidases (POX), and (iii) directly from the transformation of ozone O3. Finally, HO- radicals originate from H2O2 in this rather acidic medium (pH around 5). [13]
2.3 ROS toxicity
- Singlet oxygen 1O2
It has a rather short life span, of the order of 3 to 4 microseconds (µs), and moves little outside its place of production, essentially chloroplastic. It is, however, highly reactive and attacks photosystem pigments, photosystem II protein D1, DNA nucleosides and membrane lipids (Figure 5). Singlet oxygen reacts with the double bonds of polyunsaturated fatty acids to form lipid hydroperoxides ROOH.
- Hydroxyl radicals HO
– They attack the double bonds of the nucleic bases of DNA, which can lead to the cutting of the DNA strands.
– Amino acids of proteins are targets of HO•, which can cause the inactivation of the active site of enzymatic proteins.
– The hydroxyl radical can also attack, by lipid peroxidation, the double bonds of polyunsaturated fatty acids of membrane phospholipids and lipoproteins (Figure 6).
Besides lipid peroxyl radicals RO2– , alkoxyl radicals RO- can be produced, in the presence of Fe2+, from lipid hydroperoxides. These highly oxidizing radicals participate in the propagation of lipid peroxidation chain reactions, leading to a decrease in membrane fluidity.
- The superoxide ion O2.–
It has a longer lifetime than the HO• radical, around one millisecond (ms), which allows it to diffuse into the cells. Its direct toxicity is however less than the previous molecular species and it is quite easily transformed into H2O2 hydroperoxide.
- The hydroperoxide H2O2
It has an even longer lifetime, up to one second, which allows it to circulate in the cell by crossing the different membranes, probably using aquaporins (see Figure 7). It is considered to be not very reactive and has a role as a signal molecule.
However, the latter two ROS are quite capable, if present in high concentrations, of oxidizing lipids and proteins. As we will see later (paragraph 3), cells must achieve a subtle balance between ROS production and destruction in order to survive, otherwise they enter a deleterious spiral leading to programmed cell death (PCD).
2.4. Mechanisms to fight against ROS
- Flavonoids and phenolic compounds are mostly represented in the vacuole.
- Β-carotene and α-tocopherol are present in the chloroplast where they mainly neutralize the singlet oxygen 1O2 but also the HO• radical.
- The two most represented antioxidant molecules are ascorbate, synthesized in the mitochondria, and glutathione, which is synthesized in the chloroplasts and cytosol. Ascorbate concentrations are globally 10 to 20 times higher than those of glutathione.
- Ascorbate is predominantly represented in the peroxisomes and cytosol, but chloroplasts and mitochondria also contain high concentrations. The apoplast contains little ascorbate, although it constitutes a first barrier to oxidative attacks.
- Glutathione is mainly present in the mitochondria but is almost absent from the apoplast.
- Ascorbate and glutathione can individually participate in ROS detoxification operations, but their essential role results from their association in the context of the ascorbate-glutathione cycle (AGC), also called the Halliwell-Asada-Foyer cycle, after the scientists who identified it (see Focus on Hydroperoxide detoxification). This mechanism, is present in chloroplasts, cytosol and mitochondria (Figure 7). This cycle detoxifies the hydroperoxide H2O2 (Figure 7).
- Catalases (CATs), especially in peroxisomes and glyoxysomes, can also dismantle H2O2 into H2O2 and O2 (Figures 4 & 7).
- Another group of enzymes plays an essential, even preliminary role, since its role is the transformation of the superoxide anion O2– into hydroperoxide H2O2. These are the superoxide dismutases (SOD), characterized by the metal present in their active site: Copper, Zinc, Iron or Manganese. They have been identified in chloroplasts (CuZn-SOD thylakoidal, Fe-SOD stromatic), in mitochondria (Mn-SOD), in peroxisomes (CuZn-SOD) and in the cytosol (CuZn-SOD) (Figure 7).
Finally, redoxins, thioredoxins (TRX), glutaredoxins (GRX) and peroxyredoxins (PRX), found in chloroplasts, mitochondria and cytosol, play an important role in detoxification processes (see Focus on hydroperoxide detoxification).
3. ROS roles in the response to abiotic stress
3.1 General diagram of ROS function
3.1.1 Impact of oxidative stress
- Membrane destructuring;
- Loss of electrolytes;
- Imbalances in carbon metabolism including reduced photosynthesis and increased respiration;
- Programmed cell death as the final step.
To counteract this scenario, plant cells are equipped, as described in the previous paragraph, with a whole arsenal of antioxidant systems, which allow the destruction of ROS in order to maintain a balance conducive to ensure cell survival.
3.1.2. Double function of ROS
However, fairly recent studies have shown that the balance between ROS production and detoxification also appears to be an essential mechanism of cell signaling which participates in the maintenance of cell homeostasis [14]. ROS would thus have a double function, as actors of a deleterious oxidative stress, but also as signal molecules activating gene transduction and activation processes, regulating the normal processes of growth and development, but also presumed to participate in cellular defense (Figure 9). These considerations have led to the proposal of the term “ROS processing systems”, which would be more precise than the term “antioxidative systems”, to describe the cellular components that interact with ROS [15].
3.2 Example of a response to oxidative stress: the ozone response of leaf cells
- The first response to aggression is avoidance, with the stomata tending to close. This response is observed during many abiotic stresses even if it seems natural in the case of drought (to avoid losing water) or ozone (to prevent the penetration of the oxidizing species ozone). This closure has negative consequences on the entry of CO2, but limits the internal production of ROS.
- Then, ROS present in the intercellular spaces attack the stomatal cells and penetrate into the apoplasm of the mesophyll cells. ROS are detoxified by the antioxidants present, mainly ascorbate.
However, these constitutive defenses are relatively weak and ROS can attack intracellular targets, such as the chloroplast carbon-fixing enzyme Rubisco.
- When ROS levels are still moderate, they act in parallel as signals and, through changes in gene expression, allow a reorientation of metabolism by promoting the synthesis of antioxidants and enzymatic proteins involved in the production of reducing power. ROS are also involved in the synthesis of phytohormones that act positively or negatively on cellular homeostasis and interact with each other.
- When ROS levels exceed the capacity of cells to deploy their adaptive arsenal, cellular structures are dismantled and cellular organelles lose their function, in a process of programmed cell death or apoptosis.
3.3. Perception of stress and cell signaling
3.3.1. General mode of operation
The stress response consists of several steps:
- Perception of stress,
- Involvement of membrane receptors,
- Mobilization of intracellular signals/messengers,
- Transduction/transmission of signals to transcription factors
- Changes in gene expression [16].
The diagram in Figure 11 reflects, in a rather simplified way, the general state of knowledge on the systems of reception and transmission of signals (see The fixed life of plants and its constraints). The ROS are clearly essential players, the hydroperoxide H2O2 being the emblematic representative because of its intracellular mobility:
- H2O2 can enter the cytosol through dedicated aquaporins [xii] called peroxyporins.
- In the cytosol, H2O2 initiates a cascade of kinases [xiii], the MAP kinases [17].
- H2O2 also acts on plasmalemma channels allowing calcium entry (3a) and on receptors embedded in the plasma membrane (3b, RLK from the English Receptor-Like Kinase).
- However, the perception of the stress signal can directly mobilize an RLK that could perceive changes in membrane fluidity caused, for example, by temperature stress (excessive cold or heat).
- Calcium and calcium-dependent kinases (CDPKs, 5a) activate an NADPH oxidase-like enzyme, RBOH (Respiratory Burst Oxidase Homolog, 5b), which produces superoxide ion in the apoplast, which is immediately converted into H2O2.
- The loop system linking H2O2, the Ca2+ channel and RBOH functions as a synergistic positive feedback mechanism.
- ABA can be derived from conjugated forms or have its synthesis stimulated by H2O2. ABA is recognized by a specific receptor in the plasma membrane and is at the origin of a mechanism linking it to the production of other phytohormones,ethylene [xv] (ET), salicylic acid [xvi] (SA) and jasmonate [xvii] (JA).
- Jasmonate can be derived from oxylipins, oxidative breakdown products of polyunsaturated fatty acids. It is rather considered as protective.
- Ethylene, is a phytohormone linked to tissue and organ senescence and cellular aging mechanisms while salicylic acid is generally associated with positive effects at low concentrations but toxic at higher concentrations.
These three phytohormones are constantly interacting and will maintain a cross-talk that will affect stress responses. If we consider ABA, its role will be decisive in the closure of stomata, which is conditioned by an outflow of anions and K+ ions causing a change in osmotic potential. But the role of ABA is also important in the cells of the chlorophyll mesophyll.
The set of protein kinase cascades will activate transcription factors [xviii] necessary for the regulation of gene expression. Gene expression will be modified (stimulation and/or repression of protein synthesis) depending on the participants of these cascades which are dependent on the intensity of the stress.
The signaling chains may show partial specificities depending on the type of abiotic stress, in particular drought, cold and salinity [19], heavy metals [20], high temperature [21] and ozone [22].
3.3.2. Adjustment of function according to stress intensity
The cellular mechanisms of stress response are very complex and the adjustments are subtle. The system must react according to the balance of power between a tolerable presence of ROS, allowing an adapted regulation with the help of signaling leading to homeostatic equilibrium mechanisms, and an excessive production of ROS overcoming the defences, disorganizing the regulation and provoking the setting up of cellular destruction mechanisms leading to apoptosis.
When the stress is of low intensity:
- ROS content is maintained at a reasonable level, due to the action of enzymatic and non-enzymatic antioxidant systems already present in the cells and their accelerated synthesis related to the role of these ROS in defense-adapted signaling.
- The privileged targets of photosynthetic organs are chloroplasts and the RubisCO is often affected by ROS, very early, even before photosystems. The synthesis of the subunits of the enzymatic protein is also repressed.
- Conversely, an increase in the degradation systems of carbohydrate compounds is often observed (respiratory metabolism), either by increasing enzymatic performance or by synthesis of key enzymes.
- The imbalance of the cellular redox state is then avoided by the increased production of reducing power, associated with all this degradative metabolism. The reducing power will also be used in antioxidant reactions with an increase in the synthesis of the associated enzymatic arsenal.
- The primary metabolism of carbon compounds production is in any case modified with less carbon assimilated and more carbon lost by respiration, which leads to a rarefaction of the total quantity of available compounds.
- In addition, to combat stress, a detour of biosynthetic pathways to secondary metabolism is also observed, leading to the production of flavonoid and phenolic compounds. These changes in carbon distribution can have a long-term negative effect on plant growth.
In addition to these responses, which are mostly common to all types of abiotic stress, some proteins are synthesized specifically according to the nature of the stress:
- Osmoprotectants such as proline and glycinebetaine [xix] (osmotic stress, including water stress),
- HSP (Heat Shock Proteins, during high temperature stress),
- Proteins against aggregation/inactivation such as LEA (Late Embryogenesis Abundant Protein) during drought or cold stress, etc….
When the stress intensifies, by increasing its mode of action (increase in temperature, ozone concentration, salt content; etc…) or when it lasts very -too- long, the balance that allows a fair balance between production and destruction of ROS is broken. The signaling is disrupted and ROS play their full toxic role by destroying the membranes, allowing the synthesis of protein-destroying enzymes, leading to cellular disorganization and organ death.
4. ROS and the signaling relationships between cellular organelles and the nucleus
4.1. Redox balance and cellular compartmentalization
- In the case of chloroplasts, the singlet oxygen 1O2 causes the oxidation of carotenoids. The product of this oxidation acts as a stress signal [23]. The outcome of this retrograde signaling from the chloroplast to the nucleus is a change in gene expression in favor of photoacclimation.
- In the case of mitochondria, abiotic stresses lead to an increase in internal ROS production at respiratory chain complexes (see Figure 4). The influx of electrons to the different elements of the respiratory chain causes an increase in the transport of electrons, which becomes difficult to channel towards the final transformation of oxygen into water and causes a bottleneck favorable to the production of superoxide ions O2–.. A backup system, consisting of a particular oxidase, AOX (alternative oxidase, see Figure 4), allows direct capture of electrons and reduction of oxygen to water without participating in the establishment of the protomotive force necessary for the production of ATP. This valve minimizes the formation of superoxide ions [24]. There is, also in this case, a retrograde signaling, from the mitochondria to the nucleus, which pushes the latter to trigger the synthesis of AOX (Figure 12).
Communication between cellular organelles, chloroplasts and mitochondria, with the nucleus, through retrograde signaling, is therefore essential to maintain intracellular energy homeostasis at least during low and medium amplitude stress (Figure 12).[25]
4.2 Memory effect and epigenetics
4.3. Systemic response
Finally, it is important to understand the means by which the abiotic stress signal perceived by one part of a plant (leaf, root) spreads throughout the plant. The transmission of the stress signal from one leaf to its neighbors addresses the need for coordination of the response of all organs of a plant to that stress (systemic response). This coordination could be achieved by a self-propagating wave of ROS production, initiated at the level of a group of leaf cells and then spreading to the rest of the plant [28].
5. Prospects for the use of knowledge on signaling networks
The question arises as to the possible use of the findings on signaling networks and, in particular, on the multitude of transcription factors that are involved. Conventional methods of breeding stress-tolerant plant varieties, although useful, are proving to have their limitations. Attempts to improve stress resistance have therefore consisted in overexpressing certain types of enzymes, for example those involved in the synthesis of osmoprotectors [xx]. But the modification of a single enzyme is not sufficient because of the multiplicity of metabolic responses to stress.
Instead, genetic engineering using regulatory proteins, such as transcription factors, could be a more attractive approach to facilitate plant tolerance to stress [29]. Indeed, a single transcription factor is capable of controlling the expression of a large number of genes and current knowledge allows linking the role of several of these transcription factors to the response of plants to abiotic stresses.
Promising trials have already taken place with the creation of transgenic plants using transcription factors, notably to improve tolerance to drought, cold and salinity. However, for this technique to be beneficial in obtaining stable and productive transgenic plants, research is still needed, particularly on the dialogue between the different components of the signaling system and on the regulation of the behavior of these transgenic plants under favorable environmental conditions.
6. Messages to remember
- Under favorable environmental conditions, plants usually produce ROS, oxidative compounds that are known to be toxic to plant cells.
- The balance between ROS production and destruction is finely regulated, using the constitutively present antioxidant system. Cellular homeostasis is ensured and, at the same time, ROS play a major role, in association with phytohormones, in the cell signaling that ensures growth and development.
- When environmental stresses occur, the production of ROS is increased causing changes in membrane status and ionic balance.
- Signaling processes, combining ROS and hormones, will have to ensure the renewal of non-enzymatic and enzymatic antioxidant systems. A reorganization of the metabolism then follows from a modification of the expression of the genes, controlled by the signals coming from the cytosolic transmission cascades (messengers, kinases, transcription factors), but also carried by the organelles supplying energy, chloroplasts and mitochondria.
- The ROS-dependent stress signal is transmitted from cell to cell and then, in a systemic way, to the whole plant, so that an adapted response is proposed.
- The adjustments resulting from this intracellular mobilization may not be sufficient during more intense or prolonged stresses and the backup mechanisms may be overwhelmed. Membrane disorganization due to uncontrolled ROS attack is accompanied by disruptions in ionic balance and energy homeostasis, leading to a deleterious spiral leading to programmed cell death.
- A memory effect seems to be a decisive advantage allowing a plant having undergone a stress to respond with more efficiency to the reiteration of this stress.
- Genetic engineering could allow the construction of transgenic plants overexpressing transcription factors involved in the signaling and expression of adaptation genes, thus improving stress tolerance. In the current context of increasing adverse environmental conditions on plants, these techniques would be likely to maintain their productivity at an acceptable level.
Notes and captions
Cover image. This plant, Welwitschia mirabilis, grows in Namibia in a hot, dry environment. Photo P. Dizengremel
[1] As opposed to biotic stresses resulting from the deleterious action of a living organism on another living organism such as an attack by a pathogen (bacteria, fungi, insects, etc.)
[2] Dizengremel P (2021) Ozone and plants, the history of a toxic relationship. The Conversation, May 2, 2021.
[3] Cernusak LA, Haverd V, Brendel O, Le Thiec D, Guehl JM & Cuntz M (2019) Robust response of terrestrial plants to rising CO2. Trends in Plant Science, 24, 578-586.
[4] Boretti A & Florentine S . (2019) Atmospheric CO2 concentration and other limiting factors in the growth of C3 and C4 plants. Plants, 8, 92 DOI:10.3390/plants8040092
[5] Dietz KJ, Zörb C & Geilfus CM (2021). Drought and crop yield, Plant Biology, 23, 881-893.
[6] Ainsworth EA, Yendrek CR, Sitch S, Collins WJ & Emberson LD (2012). The effects of tropospheric ozone on net primary productivity and implications for climate change. Annual Review of Plant Biology 63, 637-661. DOI:10.1146/annurev-arplant-042110-103829.
[7] Ainsworth EA, Lemonnier P & Wedow JM (2020). The influence of rising tropospheric carbon dioxide and ozone on plant productivity. Plant Biology 22, 5-11.
[8] Matyssek R, Le Thiec D, Löw M, Dizengremel P, Nunn AJ & Häberle KH (2006). Interactions between drought and O3 stress in forest trees. Plant Biology, 8, 11-17. DOI 10.1055/s-2005-873025.
[9] Das K & Roychoudhury A (2014). Reactive oxygen species (ROS) and response of antioxidants as ROS-scavengers during environmental stress in plants. Frontiers in Environmental Science, 2, 53. doi: 10.3389/fenvs.2014.00053.
[10] Hydrogen peroxide is best known by its aqueous formulation: hydrogen peroxide.
[11] https://fr.wikipedia.org/wiki/B%C3%AAta-oxydation
[12] Often called Rboh, for respiratory burst oxidase homolog
[13] Note that HO- production is also possible in the chloroplast lumen and in the mitochondrial intermembrane space.
[14] Waszczak C, Carmody M & Kangasjärvi J (2018). ReactivOxygen Species in Plant Signaling. Ann. Rev. Plant Biol., 69, 209-236.
[15] Noctor G, Reichheld JP & Foyer C (2018). ROS-related redox regulation and signaling in plants. Semin. Cell Dev. Biol. 80, 3-12. doi: 10.1016/j.semcdb.2017.07.013.
[16] Paes de Melo B, Carpinetti PdA, Fraga OT, Rodrigues-Silva PL, Fioresi VS, de Camargos LF & Ferreira MFdS (2022). Abiotic stresses in plants and their markers: A practice view of plant stress responses and programmed cell death mechanisms. Plants, 11, 1100.
[17] MAP Kinases or Mitogen-Activated Protein Kinases.
[18] Kumar M, Kesawat MS, Ali A, Lee SC, Gill SS & Kim HU (2019). Integration of abscisic acid signaling with other signaling pathways in plant stress responses and development. Plants, 8, 592. doi:10.3390/plants8120592.
[19] Boudsocq M & Laurière C (2005). Osmotic signaling in plants. Multiple pathways mediated by emerging kinase families. Plant Physiol, 138, 1185-1194. DOI : 10.1104/pp.105.061275.
[20] Berni R, Luyckx M, Xu X, Legay S, Sergeant K, Hausman JF, Lutts S, Cai G & Guerriero G (2019). Reactive oxygen species and heavy metal stress in plants: Impact on the cell wall and secondary metabolism. Env. Exp. Bot. 161, 98-106.
[21] Mansour A (2008). Heat schock activation of phospholipase C signaling pathway in tobacco cells. Plant Stress, 2, 131-137.
[22] Hasan MM, Rahman M, Skalicky M, Alabdallah NM, Waseem M, Jahan MS, Ahammed GJ, El-Mogy MM, El-Yazied AA, Ibrahim MFM & Fang XW (2021) Ozone induced stomatal regulations, MAPK and phytohormone signaling in plants, International Journal of Molecular Sciences, 22, 6304. https://doi.org/10.3390/ijms 22126304
[23] Shumbe L, D’Alessandro S, Shao N, Chevalier A, Ksas B, Bock R & Havaux M (2017) METHYLENE BLUE SENSITIVITY 1 (MBS1) is required for acclimation of Arabidopsis to singlet oxygen and acts downstream of β-cyclocitral Plant, Cell and Environment, 40, 216-226. doi: 10.1111/pce.12856.
[24] Gandin A, Dizengremel P &Jolivet Y (2021). Integrative role of plant mitochondria facing oxidative stress: the case of ozone. Plant Physiol. Biochem, 159, 202-210.
[25] Noctor G & Foyer C (2016). Intracellular redox compartmentation and ROS-related communication in regulation and signaling. Plant Physiol. 171, 1581-1592. doi/10.1104/pp.16.00346.
[26] Ding Y, Fromm M & Avramova Z (2012) Multiple exposures to drought ‘train’ transcriptional responses in Arabidopsis. Nat. Commun. 3:740 doi: 10.1038/ncomms1732.
[27] Kinoshita T & Seki M (2014) Epigenetic memory for stress response and adaptation in plants. Plant Cell Physiology 55, 1859-1863. doi:10.1093/pcp/pcu125.
[28] Choudhury FK, Rivero RM, Blumwald E & Mittler R (2017). Reactive oxygen species, abiotic stress and stress combination. Plant Journal, 90, 856-867. doi: 10.1111/tpj.13299.
[29] Lakra N, Nutan KK, Singla-Pareek SL & Pareek A (2013). Modulating the expression of transcription factors: an attractive strategy for raising abiotic stress tolerant plants. Plant Stress, 7, 84-99.
The Encyclopedia of the Environment by the Association des Encyclopédies de l'Environnement et de l'Énergie (www.a3e.fr), contractually linked to the University of Grenoble Alpes and Grenoble INP, and sponsored by the French Academy of Sciences.
To cite this article: DIZENGREMEL Pierre (June 5, 2023), Environmental constraints and oxidative stress in plants, Encyclopedia of the Environment, Accessed July 27, 2024 [online ISSN 2555-0950] url : https://www.encyclopedie-environnement.org/en/life/environmental-constraints-oxidative-stress-plants/.
The articles in the Encyclopedia of the Environment are made available under the terms of the Creative Commons BY-NC-SA license, which authorizes reproduction subject to: citing the source, not making commercial use of them, sharing identical initial conditions, reproducing at each reuse or distribution the mention of this Creative Commons BY-NC-SA license.