Thunderstorms: electricity in the air
PDF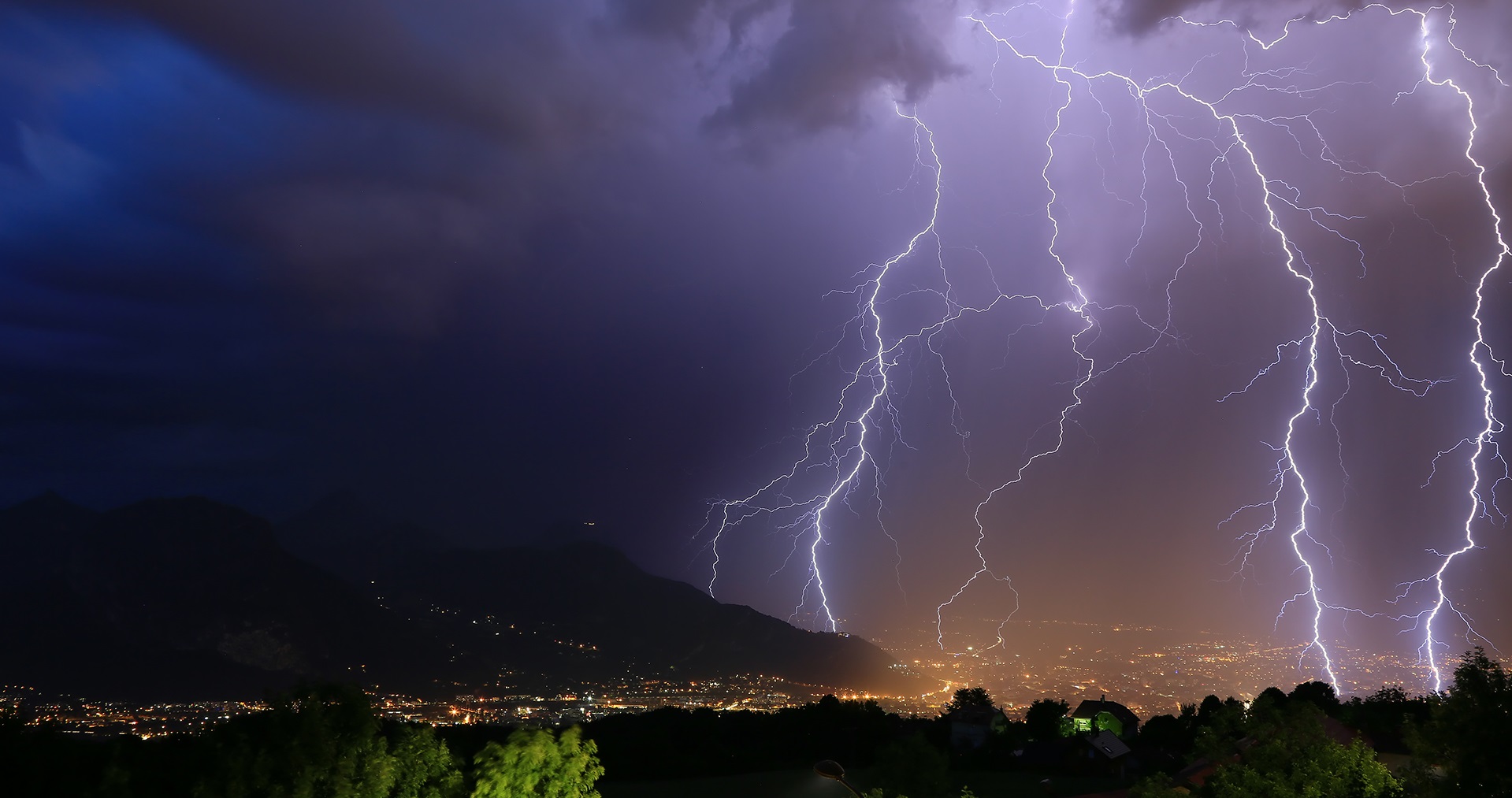
Thunderstorms are made up of clouds called cumulonimbus. A combination of phenomena (updraft of moist and warm air, condensation, evaporation, downdrafts of cool air) causes intense precipitation, wind gusts, lightning and thunder, which can impress by their violence. Let us take a closer look at what governs these phenomena and their consequences.
1. What is a thunderstorm?
2. How does a thunderstorm work?
In the lower layers of the atmosphere, the air is mixed. The air parcels move up and down with the turbulence. The pressure of air parcels that rise in the cumulonimbus gradually decreases as the ambient pressure becomes lower and lower (see article The atmosphere and the Earth’s gaseous envelope). Since they exchange little heat with their environment, their pressure decrease is adiabatic (link to article “Thermodynamics and entropy”), which leads to cooling. They can then reach conditions of temperature and pressure that cause water condensation. This change of state, however, requires the presence of germs (aerosols or micro droplets already formed), around which the water molecules initially dispersed among the nitrogen and oxygen molecules that are the main components of dry air come together. This is how the cloud is formed, usually within the first few hundred metres of upward motion. At higher altitudes, when the temperature falls below 0°C, condensation can occur directly as ice crystals. However, droplets may remain in a liquid state, in a supercooling state, up to temperatures of -40°C.
Condensation releases latent heat (unlike evaporation, which causes cooling). Thus, while the temperature drop in the troposphere (see The Earth’s atmosphere and gaseous envelope ) is on average about 6.5°C per kilometre, in the ascent of cumulonimbus it can be only 5°C per kilometre. This helps to make the rising air warmer and lighter than its environment. Under the influence of Archimedes’ force of buoyancy, this air continues to rise to the altitude where the cumulonimbus air parcels are as dense as their surroundings. This explains the large vertical extension of cumulonimbus clouds to altitudes of 10 or 15 km, i.e. to the upper limit of the troposphere. As they reach the stratosphere, the rising particles encounter higher temperatures, and therefore lighter air, which results in negative buoyancy and stops the vertical development of the cloud. Since upward motions continue below, the cloud spreads horizontally forming the anvil visible in Figure 1. The dark aspect of cumulonimbus [2] is that this thick cloud reflects and absorbs sunlight which diminishes the amount of solar radiation arriving at surface level (see The colors of the sky).
At the beginning of convective ascent, micro-droplets may rise or remain suspended as long as their fall speed is less than the updraft velocity of the air. On the other hand, the largest and heaviest drops, formed as a result of collisions and coalescences of smaller drops, fall and form rain. Similarly, the most developed ice crystals form snowflakes, which can return to a liquid state during their fall at altitudes where the temperature returns to above 0°C. Hail is formed by icing as a result of repeated collisions between ice particles and droplets of supercooled water. It is also remarkable that some hail circulates several times in the convective loop, up to the top of the troposphere where the temperature drops to less than -40°C, alternating phases of crystal agglomeration, freezing and icing, as well as partial remelting, which can lead to an internal structure in concentric layers similar to onion peels.
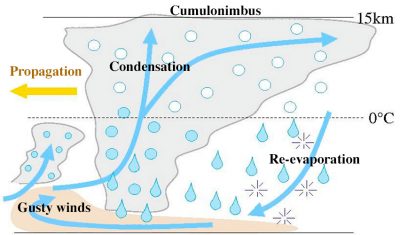
The drier ambient air of the middle troposphere, at altitudes between 4 and 8 km, interacts with the precipitation that is present on the periphery of this cloud mass. This leads to partial evaporation of the falling drops, which cools and weighs down the surrounding air, forming unsaturated descents [3], i.e. cold and heavy descending air currents (Figure 3). When they reach the ground, these currents spread horizontally, creating winds called density currents. These can generate strong and sudden gusts, which are warning signs of the storm. As shown in Figure 3, these cold and heavy air masses can in turn lift the potentially unstable air initially present in the lower level to form new ascents. This is how a thunderstorm spreads, causing new ascents downstream of the density currents. When the intensity and direction of the ambient wind varies sufficiently with altitude, the thunderstorm circulation can acquire a marked eddies characteristic, resulting in the production of tornadoes (see Tornadoes, powerful devastating eddies).
3. Conditions for thunderstorms
Once the functioning of the storm is understood, the conditions conducive to its development can be deduced. In the lower layers, processes that contribute to the lifting of air parcels, such as wind gusts in density currents, or sea breezes and mountains, are favourable to the initiation of thunderstorms.
Once an air parcel is lifted, the atmosphere must still be unstable enough to continue to rise. This instability is due to the fact that the rising air parcel is warmer and therefore less dense than its environment. In practice, the atmosphere is more unstable when the air near the ground surface is heated, particularly by sunlight. This is why, on the continents, sunny summer days are favourable to the development of storms. In addition, for rising air to form a cloud, it must contain sufficient moisture to release latent heat through condensation in liquid droplets and ice crystals.
4. Lightning and thunder
The best known of the light signatures of a storm is lightning. It results from the ionization of the air in a scenario that can be summarized as follows [4]. Collisions between different ice particles are accompanied by exchanges of electrical charges whose polarity depends on temperature. If it is cooler than -15°C, the smallest particle (an ice crystal) carries a positive charge while the largest (frosted aggregate or ice pellet) carries a negative charge. If the temperature is warmer than -15°C, the polarity is reversed: the small crystal carries a negative charge, then the large particle carries a positive charge. As small crystals are transported to altitude by updrafts while larger crystals fall to the surface, sedimentation of the charges gradually occurs. The resulting overall electrical structure is essentially bipolar, with a negative charge in the central part of the storm where the temperature is between -15 and -40°C, and a positive charge towards the top where the temperature is below -40°C. The structure can also be tripolar, with a secondary positive charge towards the cloud base where the temperature is above -15°C.
The progressive separation of electrical charges produces an electric field in the cloud of increasing intensity, up to values greater than 100 kilovolts per meter (100 kV/m). As the electric field hardly enters the water, it bypasses the hydrometeors present in the air (liquid water drops or ice particles) and strengthens in their vicinity, much like an obstacle on a traffic lane causes an accumulation of vehicles. When the intensity of the electric field thus exceeds a few hundred kV/m, the air becomes locally conductive and small sparks carrying electric charges spontaneously develop from the ends of the hydrometeorites. This is the Corona effect [5] or peak effect, which also manifests itself in the St. Elmo Fires, small electrical discharges that appear at the ends of ship masts or aircraft wings in stormy weather.
These small sparks are grouped into precursors, a few meters long, which develop in the cloud by jumps of a hundred meters, covered in a few microseconds. As they progress sporadically, they trace the characteristic shape of lightning with multiple branches. When precursors with opposite polarities charges meet, the resulting sudden neutralization produces a powerful electric current that flows through the ionized channel and brings it to temperatures above 10,000°C. The channel then shines with very bright light and also emits strong radiation in the radio wave band. This high warming causes air to expand faster than the speed of sound, resulting in a shock wave like the bang of an aircraft crossing the sound barrier. This energy then spreads like a sound wave, it’s thunder. Since the speed of sound at 340 m/s is about one million times slower than that of light, the time difference T between the visual observation of lightning and the hearing of thunder makes it possible to estimate the distance D to D (km) ≈ T (s) / 3.
Some sufficiently energetic precursors continue their jerky propagation out of the cloud, causing an increase in the electric field at their front end by peak effect. At the surface, the reinforced field can induce the same type of sparks and precursors that develop upward from various pointed structures (tree or building tops, mountain peaks, even umbrellas, golf clubs or ice axes, etc.). When the precursor from the cloud meets a precursor rising from the surface, the neutralization of the thunderstorm electrical charge towards the ground causes a cloud-to-ground lightning, such as those often visible at the tip of the Eiffel Tower or on crosses placed on high mountain peaks. The neutralization of electrical charges, inside the cloud or between the cloud and Earth, is rarely complete. Further neutralizations can be repeated up to ten times in a row within the ionized channel that has been formed. These are the return arcs whose total duration is less than a second, and which can be visually identified by the thrilling nature of many flashes.
A lightning bolt can be represented as a conductive channel from one hundred metres to ten kilometres long, a few centimetres wide. The difference in potential between its ends is a few tens of millions of volts, and the intensity of the electric current flowing through it exceeds a thousand amperes. The power released in a flash is on average 10 to 100 billion watts (Gigawatts – GW), more than that produced by a nuclear reactor. But the very short duration of the discharges (a few tenths of a second) and the very sporadic nature of the lightning flashes, both in space and time, make the dream of solving our energy problems by producing electricity from storms illusory.
5. Storm Interactions with Atmospheric Conditions
In some regions, such as the Intertropical Convergence Zone (ICTZ) (see Atmospheric Circulation and The Key Role of Trade Winds), convective cumulonimbus clouds can occur over large horizontal areas (several hundred km). Outside the clouds, where the sky is clear, the air, like any body, emits infrared radiation (link to the article Thermal radiation). It thus loses energy, cools down and thus descends at speeds less than 1 cm/s. In contrast, upward motions up to 30 m/s are concentrated in the central part of the thunderstorms [9] (Figure 5).

There is also an amplification effect between storms and large-scale atmospheric circulation called positive feedback [10]. Thunderstorms are favoured in areas of large-scale uplift, where moisture converges. In return, the release of the latent heat they induce helps to lighten the air column, thus reducing surface pressure and promoting large-scale air convergence (Figure 5). This amplification explains why convection is generally organized on a large scale, in the form of large clusters.
Thunderstorms also have a fundamental role in the transport of energy and water vapour in the atmosphere. They heat the air aloft by releasing latent heat, and cool it in the lower layers by partial evaporation of rain. They therefore have a stabilizing role on the temperature profile. In the anvil of the convective column, water vapour and some of the ice crystals in the rising air are expelled out of the cloud, moistening the upper troposphere and lower stratosphere. Humidity in the stratosphere plays an important role in the greenhouse effect and in the balance of the ozone layer.
Notes and references
Cover photo. [©Alain Herrault, Diverticimes (www.diverticimes.com)]
[1] Houze RA. (1977). Structure and dynamics of a tropical squall line system. My. Weather Rev. 105: 15401567.
[2] Craig F. Bohren (1997). Les nuages noirs, La Météorologie, 19: 49-51.
[3] Zipser, E. J. (1977). Mesoscale and convective-scale downdrafts as distinct components of squall-line structure. Monthly Weather Review, 105(12), 1568-1589.
[4] Roux, F. (1991). Thunderstorms. Editions Payot.
[5] https://wikipedia.org/wiki/Effet_corona
[6] Soula, S. and van der Velde, O. (2009) Transient light phenomena over storms: observation and production conditions. Meteorology, 64:20-31.
[7] Soula, S., Huet, P., van der Velde, O., Montanya, J., Barthe, B. and Bór, J. (2012). Of the jand giant ones above an isolated storm near Reunion Island. Meteorology, 77:30-40.
[9] Emanuel, K. A., David Neelin, J., & Bretherton, C. S. (1994). On large-scale circulations in convecting atmospheres. Quarterly Journal of the Royal Meteorological Society, 120(519), 1111-1143
[10] Risi, C. and Duvel, J.-P. (2014). The omadden-Julian scillation, the main mode of intra-seasonal variability in the tropics. Meteorology, 86: 57-65.
The Encyclopedia of the Environment by the Association des Encyclopédies de l'Environnement et de l'Énergie (www.a3e.fr), contractually linked to the University of Grenoble Alpes and Grenoble INP, and sponsored by the French Academy of Sciences.
To cite this article: RISI Camille, ROUX Frank (July 16, 2019), Thunderstorms: electricity in the air, Encyclopedia of the Environment, Accessed July 27, 2024 [online ISSN 2555-0950] url : https://www.encyclopedie-environnement.org/en/air-en/thunderstorms-electricity-in-the-air/.
The articles in the Encyclopedia of the Environment are made available under the terms of the Creative Commons BY-NC-SA license, which authorizes reproduction subject to: citing the source, not making commercial use of them, sharing identical initial conditions, reproducing at each reuse or distribution the mention of this Creative Commons BY-NC-SA license.